by John Hewitt , Medical Xpress
Although most neurotransmitters perform the same basic role, they are not fungible. Each takes the baton from an incoming action potential and passes the neural message across the synaptic divide, yet each flavor of transmitter adds its own unique twist. Simply put, they have completely different metabolic actions. Properly functioning brains require the eclectic services of all of them, everywhere, all the time. In nearly every remote outpocket of the brain, whether retina, olfactory bulb, or even transmitter-specific projection nuclei of the brainstem, all of the major transmitter systems can be found within each.
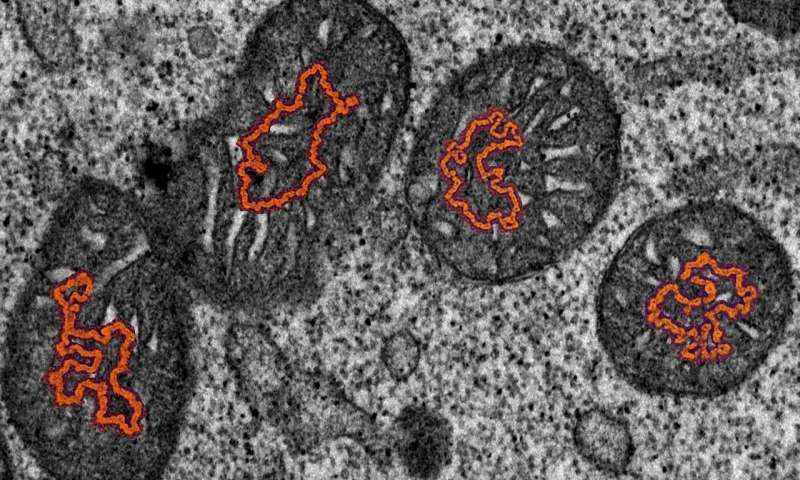
Parkinson’s disease is a devastating failure of dopaminergic transmission. One need look no further then a diseased basal ganglia—an entire neural ecosystem built in no small part on the back of dopamine—to see the devastating effects of any significant perturbation to its circuitry. Yet despite countless studies, we still have no idea what dopamine actually does for a brain, let alone for a single synapse. In other words, what makes it irreplaceable?
Provided they are properly harnessed, dangerous reactions can also be useful reactions in biology. There have been plenty of mutterings about why dopamine neurons are uniquely susceptible to oxidative damage. The usual suspects, mitochondria and redox reactions, are typically to blame. However, a complete chain of events that intimately connects synaptic transmission to processes that power it has yet to emerge.
In this void, a recent paper published in Nature Neuroscience provides a tantalizing new synthesis for dopaminergic transmission: Dopamine metabolized by monoamine oxidase (MAO) localized to the outer mitochondrial membrane furnishes electrons directly to the electron transport chain. In order to do this, electrons would have to traverse the outer membrane somehow, then the intermembrane space, and ultimately, enter the inner membrane compartment. The presumed reaction mechanism for MAO is to oxidize transmitters by replacing their amino group with a single oxygen from water. It does this along with the help of its covalently bound flavin cofactor FAD, which is converted into FADH in the process. FAD can exist in four redox states and is a versatile shuttle for electrons and protons.
In order to be regenerated, FADH is believed to react with molecular O2 to form FAD and hydrogen peroxide in the cytosol. However, the authors did not see any increase of H2O2 using their cytosolic sensor. In an accompanying commentary, it is suggested that H2O2 could somehow be localized to mitochondria, and could donate electrons to a soluble acceptor in the intermembrane space. From there, they could transfer to molecular oxygen at respiratory complex IV. .
Jim Surmeier, corresponding author on the original paper, suggested to me that this intermembrane space carrier could be cytochrome c, but did not mention H2O2 specifically. So does this mean that the FADH is leaving MAO before making H2O2? It is apparently hard to tell from these results.
To get a better handle on the many kinds of electron carriers at the table, here, we’ll take a quick look at a slightly different inner membrane enzyme system, succinate dehydrogenase or respiratory complex II. Succinate dehydrogenase also converts FAD to FADH, but it immediately transfers its electrons through iron-sulfur cluster to ubiquinone (Q) to form QH2. QH2 then ferries electrons to complex III. This is the more familiar way to go. Complex I does something similar, only it uses NAD in the initial steps before generating QH2. In fact, several other key inner membrane proteins including DHODH (Dihydroorotate dehydrogenase), ETF (Electron-transferring flavoprotein), and GPDH (Glycerol-3-phosphate dehydrogenase), all send electrons to complex III using a soluble QH2 carrier.
In their experiments, the authors short-circuited all these electron pathways by blocking complex III. They also blocked the adenine nucleotide transporter to prevent cytosolic ATP from driving complex V in reverse. Using these manipulations, they found that dopamine was able to restore the electric potential across the mitochondrial inner membrane. From this, they conclude that reactive oxygen species generated from MAO breakdown of dopamine was therefore restoring complex IV function to increase production of ATP. When the authors blocked MAO and stimulated cells with a single pulse, they found that dopamine release was unaffected. It was only after delivering trains of multiple stimuli to elicit sustained activity that the levels of ATP and dopamine release were diminished.
In the absence of MAO activity, it was generally believed that dopamine would build up in the presynapse and invariably auto-oxidize to form toxic quinones. While possible, there are many other things controlling levels of dopamine, and for that matter, any transmitter. Speaking more generally, all synapses tend to follow some variant of a very basic plan of operation. First, you release bucket loads of transmitter into the synaptic cleft. Then one of two things happens: Either the transmitter gets modified by enzymes outside the synapse—the spoils of which are fought over by both the presynaptic and postsynaptic sites—or else both sites immediately re-uptake the unmodified transmitter and process it chemically according to their own needs.
Those needs are the needs of their mitochondria. When the presynapse is poised to recoup the lion’s share of unmodified transmitter, there is a second internal struggle that pits the needs of mitochondria against the demands of the vesicle release machinery for fresh transmitter. These are the simple ground rules of the game, the underlying logic upon which the synaptic biocomputer is built. It is a catch-and-release program run ad infinitum, continually modified by various levels of feedback and feedforward signals represented by different transmitter metabolite abundances. To fully conceive of why things have apparently been designed this way, we have to take stock of what each of these products mean for the mitochondria present at both ends for each of our different transmitter systems.
For the case of gaba, we have already observed an essential activity in controlling the levels of nucleotides made available to mitochondria for replication and DNA repair. Nucleotides are serially unlocked from their cyclic forms at all G-protein-based metabotropic receptors, and further made available via gaba transaminase function. Gaba can be synthesized via the B form of Mao inside astrocytes, and gaba’s carbon skeleton ultimately enters the mitochondrial TCA cycle in synapses through the gaba shunt.
Similarly, for serotonin, there is an essential role in genesis of new mitochondria. In turn, serotonergic transmission is controlled by nucleotide transport in mitochondria.
The excitatory transmitter glutamate directly participates in transport of reducing equivalents (NADH) into neuronal mitochondria via interlinks with the malate-aspartate shuttle. It directly shuttles through astrocytes via the glutamate-glutamine cycle and mitochondrial glutamate dehydrogenase. The transmitter acetylcholine is synthesized from choline and acetyl CoA made locally in synaptic mitochondria. It is degraded in the synaptic cleft by one of the fastest enzymes known to generate a significant extracellular proton current. The supplied choline is also a contributor to fatty acid metabolism and membrane lipid synthesis for the establishment and maintenance of myelin.
The linking of the catecholaminergic ecosystem to critical mitochondrial electron transport functions here completes our ground-floor-level understanding of all major neurotransmitter families. While it does not directly explain higher-order features seen in dopaminergic circuits across the evolution of the species, it is tempting to speculate on a few conserved aspects of synaptic structure. For example, neurons in our dopaminergic systems are characterized by the ubiquitous presence of symmetrical invertebrate-style “dendro-dendritic” synapses. By contrast, most other regions of our brains deploy polarized asymmetric junctions where one side has the vesicles and the other side the postsynaptic density.
One thing that differentiates our symmetric synaptic regions is that we have tweaked the catecholaminergic circle of transmitter friends away from invertebrate ‘octopamine’ toward dopamine. With the advent of new connectome-generating technology like flybrain, we can now read out the structural effects that correspond to chemical changes. If fact, it is so easy to do now that machines can be programmed to do it automatically. For example, AI recognition algorithms can pick out every signature ‘polyadic T-bar’ release site found in every synapse in the entire brain of a drosophila. When we can do this for human brains, our remaining transmitter mysteries will evaporate.
Leave a Reply